Hemocompatibility of Albumin Nanoparticles as a Drug Delivery System—An in Vitro Study ()
Received 20 February 2016; accepted 5 April 2016; published 8 April 2016

1. Introduction
Nanoparticles, liposomes, and micelles have received a considerable amount of attention by researchers as drug delivery carriers for site-specific drug delivery. The recent advances in nanoparticles in drug targeting have resulted in a new focus on the role of nanoparticles in disease management. Nanoparticles were first prepared using non-biodegradable polymers such as polyacrylamide, polymethyl methacrylate and polystyrene [1] . However, they could not be considered for systemic use due to the toxicity caused by the non-biodegradable property of the polymer. Hence, biodegradable polymers such as polycyanoacrylate have been used for the preparation of nanoparticles [2] . Nanoparticles have recently received much attention due to their versatile nature, particularly in the field of disease diagnosis, prevention and treatment at the cellular and molecular levels. Nanoparticles can be administered by oral and intravenous routes. In the oral route, they are generally used to increase the oral bioavailability of proteins, peptides and vaccines [3] . In the intravenous route, they are used for the site-specific delivery of a wide range of drugs such as antibiotics to treat intracellular infections and oligonucleotides to protect them from enzymatic degradation and to enhance their penetration across biological membranes. Nanoparticles are useful to deliver several drugs into the brain, including anti-cancer and anti-Alzheimer’s drugs [4] . Hence, the usage of nanoparticles in disease management provides tremendous hope in the treatment of many deadly diseases such as cancer, AIDS, intracellular infections and cardiovascular diseases.
Albumin is the most abundant protein in plasma (35 - 50 g/l human serum) with a molecular weight of 66.5 kDa. It is stable in the pH range of 4 - 9, soluble in 40% ethanol, and it can be heated at 60˚C for up to 10 h without deleterious effects. These properties, as well as its preferential uptake in tumors and inflamed tissue, widespread availability, biodegradability, and lack of toxicity and immunogenicity make serum albumin an ideal candidate for drug delivery. Recent applications of serum albumin (human and bovine) have demonstrated certain of its advantages as a natural and therefore biocompatible and biodegradable carrier for drugs [5] [6] . The use of albumin for preparing biodegradable nanoparticles has gained great interest in therapeutics, particularly in cancer treatments. Because the carriers deliver the drugs in a controlled manner to the targeted cancer cells, a lower amount of drug would be sufficient to obtain the therapeutic effect, which consequently minimizes the side effects. Albumin contains amino and carboxylic groups, and these groups can be used for surface modifications. Moreover, human serum albumin-based nanoparticles have been well tolerated without any serious side effects in clinical trial studies [7] [8] . Nanoparticles of minimal size can enhance the efficacy of drug metabolism with minimal side effects because they allow for the possibility of site-specific targeted delivery [9] [10] .
Albumin-based nanoparticle carrier systems represent an attractive strategy because a significant amount of drug can be incorporated into the particle matrix due to the different drug binding sites that are present in the albumin molecule [11] . Because of the defined albumin primary structure and high content of charged amino acids (e.g., lysine), albumin-based nanoparticles could allow the electrostatic adsorption of positively (e.g., ganciclovir) or negatively charged (e.g., oligonucleotide) molecules without the requirement of other compounds [12] [13] . In addition, albumin nanoparticles can be easily prepared under soft conditions by coacervation, controlled desolvation or emulsion formation. They have a smaller size (50 to 300 nm) compared with microparticles and, in general, they have better controlled release properties than liposomes which may improve patient acceptance and compliance. Commercially, albumins are obtained in significant quantities from egg white (ovalbumin), bovine serum (bovine serum albumin, BSA), and human serum (human serum albumin, HSA) and also available from soybeans, milk, and grains [14] .
Hemocompatibility is a very important factor to consider when deciding on the application of implantable biomaterials such as artificial blood vessels and orthopedic implants. With the development of blood-contacting materials or implantable devices, it is necessary to improve the hemocompatibility by surface modification or re-design. When blood contacts a foreign biomaterial surface, the first process that occurs is the competitive adsorption of plasma proteins [15] . Many studies have aimed to improve hemocompatibility of biomaterials by surface modification [16] [17] . The improvement of hemocompatibility on a biomaterial surface aims at reducing protein adsorption with the eventual goal of decreasing platelet adhesion
According to the variety of the applications of albumin nanoparticles, the present work aims to prepare bovine albumin nanoparticles (BAN) using the simple coacervation method. To evaluate the interaction between synthetic BAN and human blood, hemocompatibility tests were performed in vitro.
2. Materials and Methods
2.1. Chemicals
Bovine albumin lyophilized powder (A2153 SIGMA), 0.5 M sodium hydroxide, and 4% glutaraldehyde. The chemicals used were analytical grade.
2.2. Albumin Nanoparticle Preparation
Bovine albumin was dissolved in distilled water (0.02 g/ml). The bovine albumin solution was filtered using a Millipore filter. The filtrate was placed in a beaker and adjusted to pH7.4 using 0.5 M sodium hydroxide solution. The solution was stirred on a magnetic stirrer, and the required amount of acetone was added dropwise from a syringe until the solution became turbid. BAN were cross-linked by adding 100 µl of a 4% glutaraldehyde-ethanol solution, and the solution was mixed continuously for 3 h at room temperature. After the cross-linking stage, BAN were again filtered through the Millipore membrane filter. The filtrate was centrifuged at 20,000 rpm at 20˚C for 30 min. After centrifugation, the supernatant was removed, and the suspension was washed four times with acetone. Finally, BAN were suspended in an acetone-water mixture.
2.3. Albumin Nanoparticle Characterization
The sizes, morphology of the BAN were examined using scanning electron microscopy (SEM) (JEOL, JSM- 6360 LA Japan).
2.4. BAN Hemocompatibility Tests
Blood samples were drawn by venipuncture from 20 healthy adult human donors of the same age and gender. Blood from each donor was collected in two different evacuated tubes: one contained EDTA (1.5 mg/l) as an anti-coagulant, and the other contained sodium citrate, and both tubes contained 5 ml blood. After the gentle mixing, each blood sample was divided into six tubes. One of the tubes was used as a control without the addition of BAN.
The prepared BAN was added to blood in each tube with at the following concentrations: 0.34 mg/ml, 0.67 mg/ml, 1 mg/ml, 1.34 mg/ml, and 1.67 mg/ml. Control blood samples contained no BAN, and blood samples with BAN were incubated for 2 h at 37˚C.
2.5. Complete Blood Count (CBC)
2 ml of anti-coagulated blood incubated with BAN were sent to a clinical lab to perform a CBC. CBC analysis was also conducted on the control samples without BAN.
2.6. Hemolysis Assay
Anti-coagulated blood incubated with BAN was centrifuged and washed with phosphate buffer saline (PBS) five times in a centrifuge according to the procedure reported in literature [16] [17] to completely remove plasma and obtain erythrocytes. Erythrocytes were suspended in PBS. A 0.2 ml aliquot of the diluted suspension was transferred to a 5 ml tube that was filled with 0.8 ml of water (as a positive control) and PBS buffer (as a negative control), respectively. The supernatant was analyzed using a spectrophotometer to record the absorbance at 540 nm. The hemolytic percentage (HP) can be calculated using the following equation:
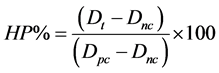
where the Dt is the absorbance of a test sample, Dnc is the absorbance of the negative control and Dpc is the absorbance of the positive control.
2.7. Prothrombin Time (PT)
200 µl of thromboplastin with calcium was incubated at 37˚C for 15 min. Plasma was separated from the blood incubated with BAN and with sodium citrate as an anti-coagulant. 100 µl of plasma at 37˚C was added to thromboplastin with calcium. The clotting time was monitored using a stopwatch.
2.8. Erythrocyte Sedimentation Rate (ESR)
Blood samples collected on sodium citrate and incubated with BAN with different concentrations were checked for clots using two applicator sticks. Using a capillary pipette, the Wintrobe tube was filled to the 0 mark with a blood sample. The tube was left in an exactly vertical position in the rack. After 60 min, the level of the erythrocyte column was recorded. This result was the erythrocyte sedimentation rate in mm/h.
2.9. Statistical Analysis
The data from the hemocompatibility tests are represented as the mean ± SD in all graphs. Chart bars diagram of the values obtained were drawn. The Pearson’s correlation coefficient (R2) was determined.
3. Results and Discussion
Human serum albumin nanoparticles containing noscapine were prepared by the coacervation method, in which ethanol was used as a dissolving agent, followed by crosslinking using glutaraldehyde [18] . The pH-coacerv- ation method has widely been used in the encapsulation of water-soluble and insoluble drugs. With the modified pH-coacervation technique reported in this study, Safaa Sebak et al. obtained noscapine-loaded human serum albumin nanoparticles with diameters between 150 and 300 nm with a narrow size distribution [19] . In the present study, the coacervation method was used to produce BAN at pH 7.4 at 37˚C. BAN obtained were in the size range of 250 to 350 nm with a mostly uniform shape as shown in Figure 1.
Many nanoparticle products have been designed for intravenous administration such as targeted and controlled drug delivery, as contrast agents for imaging or as vaccine carriers. However, the mechanism of interaction of nanoparticles with blood is not well understood. The evaluation of the compatibility of nanoparticles in blood can be critical in the design of the nanoparticle for in vivo applications. Various assays can be used to evaluate hemocompatibility including erythrocyte composure (hemolysis and hemagglutination), thrombogenicity, cytokine assays, complement activation (protein adsorption), phagocytosis, and toxicity [20] .
Erythrocytes are perfect osmometers where disturbances in osmotic and physical changes in blood can cause their lysis. Erythrocytes are also critical in maintaining proper flow characteristics in microcirculation, thus preventing vessel occlusion. In general, erythrocyte destruction can be caused by several factors such as bacterial infection, intrinsic erythrocytes membrane defects, mechanical shear due to blood flow, osmotic and pH changes in blood, drug-induced hemolysis, excessive complement, and platelet activation [21] . The exposure of erythrocytes to nanoparticles could result in hemolysis and/or hemagglutination. Because hemolysis results in the release of red-colored hemoglobin, colorimetric assays can be used to estimate the extent of damage. Nanoparticles also function as antigens in bridging erythrocytes, causing hemagglutination, which results in clumping or agglutination to form a diffuse lattice. This effect has serious consequences in humans leading to the obstruction of blood vessels, imbalance in osmolarity of blood, and the inability of erythrocytes to carry out normal functions [20] . Accordingly, the effect of BAN on erythrocytes hemolysis was analyzed. My results showed that the percentage of HP decreased as the BAN concentration increased. The correlation between BAN concentration and HP % was strong (R2 = 0.92), and they were negatively correlated. The values of HP% were in the normal acceptable range between 0% - 1% for all of the examined concentrations of BAN as indicated in Figure 2. It was shown that albumin has a preventative effect against erythrocytes hemolysis [22] [23] . The result was in accordance with the previous finding that BAN reduced the percentage of HP. As shown in Figure 3, the HG
![]()
Figure 1. Scanning electron micrographs (SEMs) of BAN prepared pH 7.4, 60 k resolution. BAN were found to be of uniform size.
![]()
Figure 2. HP% is slightly changed with BAN concentrations.
![]()
Figure 3. Ata BAN concentration of 0.67 mg/ml, the HG concentration begins to increase as the concentration of BAN increases.
(mg/dl) concentration was in the normal range, and no abnormal effect was observed due to the increase in BAN concentration. The relationship between the HG and BAN concentration was strongly positively correlated (R2 = 0.9). MCH and MCHC were strongly correlated with the BAN concentration. Increases in MCH and MCHC were obtained as the concentration of BAN was increased (Figure 4, Figure 5). For MCH and MCHC, the relationship with BAN concentration was strongly correlated (R2 = 0.98). Incubation and storage of RBCs led to an echinocytic shape transformation, which was reversible upon incubation in albumin solutions. This process was time-, concentration- and hematocrit-dependent. Treating RBC units at the end of their shelf-life by adding 20% albumin or washing them in 0.2% albumin reversed all degrees of echinocytosis towards discocytosis. In conclusion, albumin has the capacity to reverse echinocytosis generated by RBC storage [24] . Yubin Huang et al. indicated that the coexistence of a 10 - 44 vol% of rHSA-FeP did not cause any influence on the number of WBCs and RBCs during the 6-h observation period. This was further proven by the microscopic observation of the morphological change of the RBC. No deformation or aggregation of RBC was observed for the blood suspension with the 10, 20, and 44 vol% of rHSA-FeP after 6 h at 37˚C [25] . MPV was linearly related to BAN concentrations. The relationship between them was strongly positively correlated (R2 = 0.93). In accordance with previous studies, Figure 6 showed that there was no noticeable change in MPV due to the increase in BAN concentrations.
The effect of rHSA-FeP on blood coagulation was also investigated by the determination of the prothrombin time (PT) and activated partial thromboplastin time (APTT). It is well known that thromboplastin and prothrombin are common blood coagulation factors that exist in the blood serum, platelet, and WBC. For example, when a certain amount of calcium or phospholipids is added to the blood, the activated thromboplastin induces the inversion of prothrombin to thrombin, which leads to the coagulation of the blood [26] . In agreement with the previous results in Figure 7 and Figure 8, the BAN concentrations reduced PT and ESR. BAN concentration was correlated negatively with PT and ESR. A strong correlation between PT and BAN concentration (R2 = 0.88) was obtained. A strong correlation between PT and BAN concentration was also obtained (R2 = 0.92). All
![]()
Figure 4. MCH correlate positively to BAN concentration.
![]()
Figure 5. Variation in the values of MCHC is monitored as the BAN concentrations increase.
![]()
Figure 6. MPV increases linearly with BAN concentration. The plateau in MPV values at high concentrations of BAN is indicated.
of the values of the blood indices obtained in this study were in the normal range, even at a high concentration of BAN.
4. Conclusion
It can be concluded that BAN has no harmful effect when it interacts directly with blood. The results obtained in this study emphasize that BAN has a high degree of biodegradability and biocompatibility. BAN can be used as
![]()
Figure 7. Higher concentrations of the BAN were associated with faster clotting formation, but the PT remained within the normal range.
![]()
Figure 8. A notable decrease in ESR is shown at higher concentrations of BAN.
a promising tool in many biological and medical applications.
Acknowledgements
The author would like to acknowledge financial support of this work from the Deanship of Scientific Research (DSR), University of Tabuk (Tabuk, Saudi Arabia, under grant no. (S-1436-0145)).