Supplementing fibroblast growth factor 2 during bovine oocyte in vitro maturation promotes subsequent embryonic development ()
1. INTRODUCTION
Several paracrine and autocrine factors are produced within the follicular niche control oocyte maturation [1- 3] . Supplementing specific paracrine and endocrine components during in vitro maturation (IVM) of bovine cumulus-oocyte complexes (COCs) can improve the success of in vitro embryo production and maximize embryonic competency to generate healthy offspring after embryo transfer. Providing gonadotropins, namely FSH, is commonplace for maximizing COC maturation and fertilization [2,3] . Oocyte-secreted factors also improve oocyte competency. Supplementing growth differentiation factor 9 (GDF9) and/or bone morphogenetic protein 15 (BMP15) during IVM improved subsequent bovine embryo development [4], and supplementing GDF9 during IVM of mouse COCs improved embryo development and post-transfer survival [5] . Increased bovine embryo development rates were also observed after supplementation with fibroblast growth factor 10 (FGF10), a thecaderived factor [6,7] .
Several other FGFs are produced in oocytes and follicular somatic cells of cattle [6-10] , pigs [11], mice [12- 14] and women [15,16] . Also, each of the four main classes of receptors that interact with FGFs (termed FGFR1-4) is present within oocytes and granulosa and cumulus cells [6] . FGFs are involved in various biological processes during folliculogenesis, including primordial follicle activation and regulation of granulosa and cumulus cell mitosis, apoptosis and glycolysis [12,17-19] . The role of these various FGFs during the final period of oocyte maturation remain largely unexplored.
An FGF of recent interest for a functional role during oocyte maturation is FGF2 (also known as basic FGF). It is produced by theca and granulosa cells, and its transcript abundance increased during periovulatory follicle growth and during bovine IVM [20-22]. Moreover, selective FGF2 single nucleotide polymorphisms (SNPs) are linked with IVP success rate in cattle [23]. We proposed that providing FGF2 during IVM would benefit oocyte maturation in ways that benefit subsequent embryo development.
2. MATERIALS AND METHODS
2.1. Animal Assurances and Reagents
All studies were completed in accordance with and with the approval of the Institutional Animal Care and Use Committee (IACUC) at the University of Florida. Unless stated otherwise reagents were purchased from Sigma-Aldrich Co. (St. Louis, MO, USA).
2.2. Bovine in Vitro Maturation, Fertilization and Culture
The in vitro production (IVP) of bovine embryos was accomplished as described previously [6,24,25] . Briefly, ovaries were obtained from Central Beef Packing Co. (CenterHill, FL, USA) and transported to the lab. Cumulus-oocyte complexes (COCs) were collected and groups of 10 - 12 COCs were cultured in 50 μl drops of oocyte maturation medium (TCM199 containing Earle’s salts supplemented with 25 µg/ml bovine FSH [Bioniche Life Sciences, Belleville, Ontario, CA], 2 µg/mlestradiol 17-β, 22 µg/ml sodium pyruvate, 50 µg/ml gentamicin sulfate, 1 mM glutamine). In most studies serum-free maturation medium was used and 1 mg/ml polyvinyl alcohol (PVA) was included as a protein substitute. In one study, maturation medium was supplemented with 10% [v/v] bovine steer serum (Pel-Freeze Biologicals, Rogers AR USA).
After 21 h at 38.5˚C in 5% CO2 in humidified air, COCs were transferred to fertilization medium and exposed to Percoll gradient-purified bovine spermatozoa. The same pool of semen from three bulls was used throughout the studies. After 8 to10 h at 38.5˚C (5% CO2 in humidified air), cumulus cells were removed by vortexing with 1000 U/ml hyaluronidase. Denuded zygotes were placed in groups of 25 - 30 in 50 μl drops of modified synthetic oviduct medium (mSOF; Millipore Corp., Billerica, MA USA) [26]. Drops were covered with mineral oil and maintained at 38.5˚C in 5% CO2, 5% O2 and 90% N2 for 8 days.
2.3. Oocyte Maturation
Serum-free maturation medium was supplemented with 0 (vehicle only), 0.5, 5 or 50 ng/ml recombinant bovine FGF2 (R & D Systems, Minneapolis, MN USA). Stocks of FGF2 were prepared in TCM199 containing Earle’s salts (Invitrogen Corp.) and 1% [w/v] bovine serum albumin (BSA). After 21 to 22 h, COCs were vortexed for 4 min to remove cumulus cells. The proportion of oocytes that extruded their first polar body was determined by using stereomicroscopy. Seven replicate studies were completed, and each study contained from 10 to 22 COCs.
In a second study cumulus cells were removed from oocytes before IVM. For this, COCs were vortexed for 4 min and denuded oocytes were cultured in serum-free maturation medium for 21 to 22 h with 0 or 5 ng/ml FGF2. Four replicate studies were completed, and each study contained from 10 to 24 denuded oocytes.
2.4. Embryonic Development
Serum-free maturation medium was supplemented with 0, 0.5, 5 or 50 ng/ml FGF2 immediately before beginning IVM. After maturation, COCs were rinsed in PBS-PVA and were fertilized and cultured without supplemental FGF2. At day 3 post-IVF, the proportion of cleaved zygotes and embryos containing ≥8 blastomeres was recorded. The proportion blastocysts and stage of blastocyst development (regular or advanced [expanded, hatching or hatched]) was recorded at days 7 and 8 postIVF. Four replicate studies were completed, and each study contained from 26 to 35 COCs.
Blastomere numbers were determined for blastocysts at day 8 post-IVF by fixing blastocysts with 4% [w/v] paraformaldehyde, permeabilizing with 0.1% [v/v] Triton X-100 and staining with 1 µg/ml Hoechst 33342 (Invitrogen Corp., Carlsbad, CA USA) [6] . Blastocysts were mounted on slides and the number of nuclei per blastocyst was counted by using epifluorescence microscopy (Nikon TE2000, Nikon Corp., Melville, NY USA) (n = 26 to 34 blastocysts examined over 4 replicate studies).
One study used maturation medium containing serum. For this, either 0.5 ng/ml FGF2 or carrier-only was supplemented to maturation medium containing 10% bovine steer serum immediately before COC culture. The COCs were rinsed in PBS-PVA and fertilized and cultured without supplemental FGF2. Embryo development was examined at days 3, 7 and 8 and as described previously. Three replicate studies were completed, and each study contained from 25 to 34 COCs.
2.5. Cumulus Cell Expansion
Serum-free maturation medium was supplemented with 0, 0.5, 5 or 50 ng/ml FGF2 immediately before IVM. After 21 to 22 h of IVM, the degree of cumulus expansion in COCs were scored visually by phase-contrast microscopy on a 1 to 3 scale (1 = poor expansion characterized by few morphological changes compared with before maturation; 2 = partial expansion characterized by fair expansion but notable clusters lacking expansion; 3 = complete or nearly complete expansion) [6,27] . Six replicate studies were completed, and each study contained from 22 to 35 COCs.
2.6. Cumulus Cell Apoptosis
Serum-free maturation medium was supplemented with 0, 0.5 or 5 ng/ml FGF2 immediately before COC culture. The percentage of TUNEL-positive cumulus cells were determined after 21 to 22 h of maturation by using FITCconjugated dUTP and 1 μg/ml Hoechst 33342 as a counterstain (Roche Applied Sciences, Indianapolis, IN USA). COCs were mounted on slides and analyzed with epifluorescence microscopy. The proportion of TUNEL-positive cumulus cells was calculated by dividing the number of TUNEL-positive nuclei by total nuclei numbers for each COC. Four replicate studies were completed, and each study contained from 25 to 35 COCs.
2.7. Selected Transcript Profiling in Cumulus Cells
Serum-free maturation medium was supplemented with 0 or 5 ng/ml FGF2 immediately before COC culture. After 6 h of maturation or at the end of maturation (21 to 22 h), cumulus cells and oocytes were separated from each other by repeat pipetting. Pools of cumulus cellsderived from 25 to 35 COCs were transferred to microcentrifuge tubes, centrifuged at 700 × g for 2 min at room temperature to remove residual solution, snap-frozen in liquid nitrogen and stored at –80˚C. Total cellular (tc) RNA was extracted using the RNeasy Micro Kit (Qiagen, Valencia, CA USA). RNA concentrations and integrity (A260/A280 ratio ≥ 1.8) was determined using a NanoDrop 2000 Spectrophotometer (Thermo Scientific, Wilmington, DE, USA). Each tcRNAsample (10 ng/reaction) was incubated in RNase-free DNase (New England Biolabs, Ipswich, MA USA) and reverse transcribed using the High Capacity cDNA Reverse Transcription Kit (Applied Biosystems, Foster City, CA USA). One study examined a series of transcripts associated with apoptosis (BCL2, BAX, BAD, XIAP, FAS) [28,29] (n = 4 replicate studies). Another study examined several cumulus cell transcripts associated with oocyte and embryo competency (CTSB, EFGR, SPRY2, FSHR, HAS2) [6,30- 32] (n = 3 replicate studies). Primers (Invitrogen Corp.) were used at a concentration of 200 nMand were mixed with RT products and SYBR Green PCR Master Mix. After an initial activation/denaturation step (50˚C for 2 min, 95˚C for 10 min), 40 cycles of a 2-step amplification protocol (60˚C for 1 min, 95˚C for 15 sec) were completed using a 7300 Real-Time PCR System (Applied Biosystems). A dissociation curve analysis (60˚C to 95˚C) was used to verify the amplification of a single product.Each sample was run in triplicate and a fourth reaction lacking exposure to reverse transcriptase was included to verify the absence of genomic contamination. Relative amounts of 18S RNA were used as an internal control for quantifying relative gene expression. Abundance of 18S RNA did not change based on treatment. The ratio of target to reference RNA was used to determine relative expression (2–CT[target]/2–CT[reference]) [6] .
2.8. Statistical Analyses
All analyses were completed with least-squares analysis of variance (LS-ANOVA) using the general linear model (GLM) of the Statistical Analysis System [33]. All percentage data were arcsine-transformed before analysis. Percentage data are presented as non-transformed values and SEMs. Orthogonal contrasts were completed on FGF2 dose-response studies (control vs. all FGF2 treatments; 0.5 vs. 5 ng/ml FGF2; 0.5 and 5 vs. 50 ng/ml FGF2). COC expansion was analyzed by examining the mean COC expansion index (calculated for each treatment within each replicate) and by examining the proportion of COCs observed within each of the non-parametric rankings (1, 2 and 3). The ratio of target to reference RNA was used to analyze qRT-PCR data.
3. RESULTS
3.1. FGF2 Supplementation Affects Oocyte Maturation
Providing FGF2 affected first polar body extrusion (Figure 1). Specifically, an increase in the percentage of oocytes with an extruded polar body was detected when comparing controls with COCs incubated with 0.5, 5 or 50 ng/ml FGF2 (Orthogonal contrast of control vs. all FGF2 treatments; P = 0.05). No differences in polar body extrusion rates were detected between FGF2 treatments.
3.2. FGF2 Supplementation during IVM Impacts Subsequent Embryo Development
Several studies were completed to examine whether fertilization success and subsequent embryo development

Figure 1. The effect of FGF2 supplementation during IVM on oocyte maturation. COCs (n = 10 - 22/replicate; 7 replicate studies) were cultured in maturation medium containing FSH and 0, 0.5, 5 or 50 ng/ml FGF2. After 21 h, cumulus cells were removed by vortexing and the percentage of oocytes that extruded their first polar body (Panel A) and achieved MII (Panel B) was determined. In Panel A, a significant difference between controls and all FGF2 treatment groups were detected by using Orthogonal contrasts (P = 0.05). In Panel B, percentage of oocytes that reached MII differed between the control and oocytes exposed to 5 ng/ml FGF2. The other FGF2 treatments did not differ from the control.
rates were altered after COC supplementation with FGF2 (0, 0.5. 5, 50 ng/ml). In the first study, COCs were matured in medium lacking serum (replaced with 1 mg/ml PVA). As shown in Table 1, FGF2 supplementation during IVM did not affect the percentage of presumptive zygotes that cleaved at day 3 after IVF and the percentage of embryos containing ≥8 cells at day 3. Exposure to FGF2 during IVM increased the percentage of cleaved embryos that became blastocysts at day 7 (P = 0.02). A tendency for an increase in blastocyst formation at day 7 was observed when data were examined based on the percentage of oocytes that became blastocysts at day 7 (P = 0.07). No effects of FGF2 supplementation were detected at day 8 on blastocyst and advanced blastocyst rates and total blastomere number.
Since most bovine IVP systems utilize bovine steer serum in maturation medium to maximize fertilization and subsequent embryonic development potential [24,25] , a study was completed to determine if beneficial effects of FGF2 could be detected when using serum-containing maturation medium. Supplementing 0.5 ng/ml FGF2 to maturation medium containing serum did not affect the percentage of cleaved embryos or the percentage of embryos containing ≥8 cells at day 3 post-IVF (data not shown) but increased (P = 0.05) the percentage of cleaved embryos that became blastocysts at day 7 post-IVF (41% ± 3% vs. 28% ± 5% for FGF2-treated vs. control). A nonsignificant trend also was observed when data was analyzed based on the percentage of oocytes that became blastocysts at day 7 (26% ± 5% vs. 17% ± 4% for FGF2- treated vs. control; P = 0.14). Blastocyst development at day 8 was not affected by FGF2 treatment (data not shown).
3.3. Potential Role of Cumulus Cells in Mediating FGF2 Effects
Changes in cumulus cell morphology, viability, and gene expression were examined in COCs examine whether FGF2 may be acting through cumulus cells to promote oocyte maturation and improve embryo development.
Cumulus expansion rates were not altered by FGF2 supplementation. Neither mean cumulus expansion score nor the proportion of COCs within each expansion category was altered by supplementation with 0.5, 5 or 50 ng/ml FGF2 (overall averages across all treatments: 9% category 1, 67% category 2, 24% category 3; mean index average = 2.1).
The necessity of cumulus cells for mediating FGF2 effects on oocyte maturation was examined by removing cumulus cells before IVM and culturing denuded oocytes in maturation medium containing 0 or 5 ng/ml FGF2. Supplementation with FGF2 had not effect on the percentage of denuded oocytes extruding their first polar body (71% ± 9% vs. 56% ± 8% for 0 vs. 5 ng/ml FGF2, respectively). Effects of denuded oocyte culture on subsequent fertilization and embryo development were not examined.
Changes in the percentage of TUNEL-positive (i.e. apoptotic) cumulus cells were observed in COCs exposed to FGF2 treatment (Figure 2). Supplementation with either 0.5 or 5 ng/ml FGF2 decreased (P = 0.03) the percentage of TUNEL-positive cumulus cell nuclei at the end of the maturation period when compared with the control.
To follow up on this observation, the influence of FGF2 supplementation on expression profiles of several
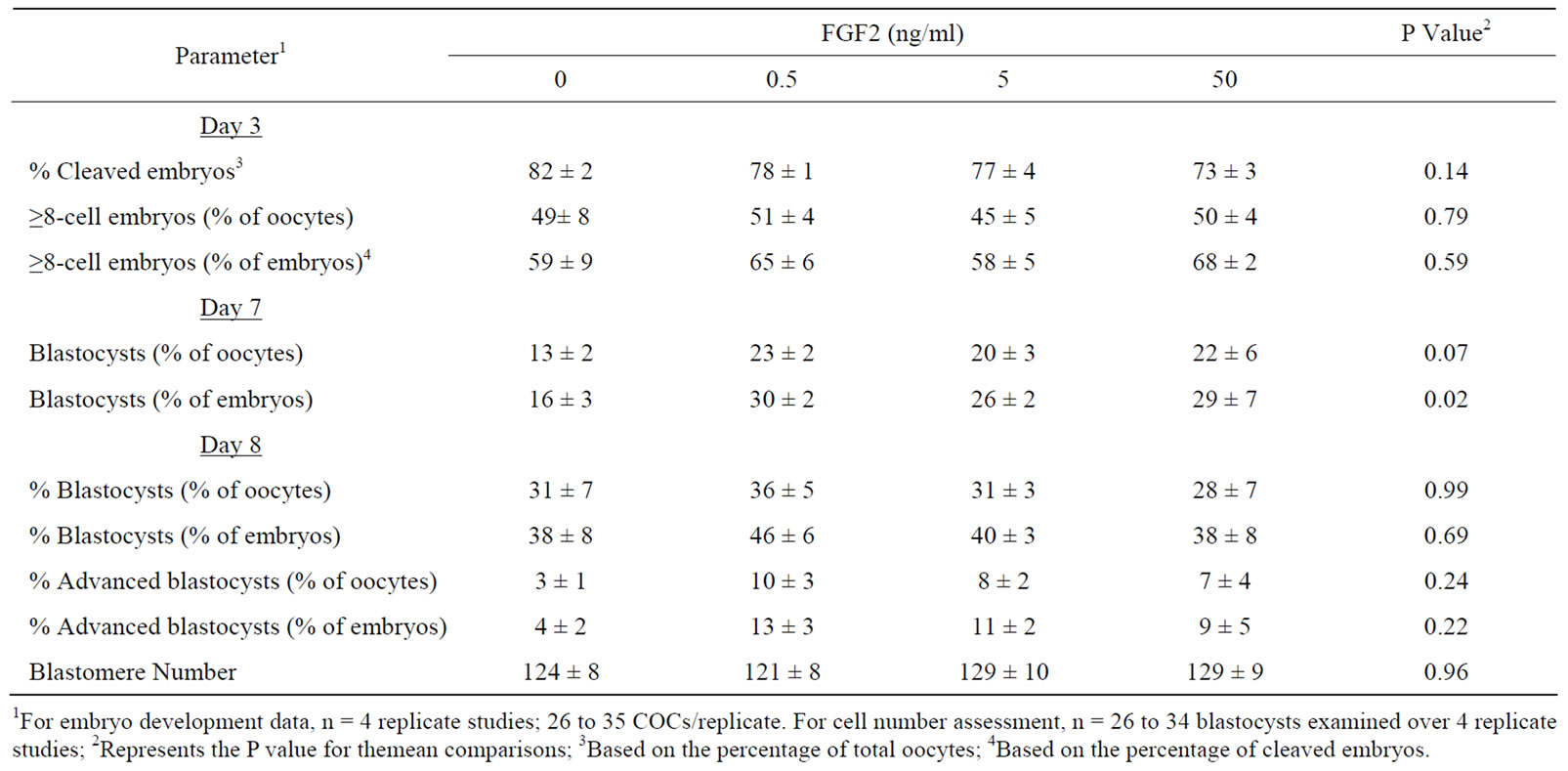
Table 1. The effect of supplementing FGF2 during COC maturation on subsequent in vitro development.
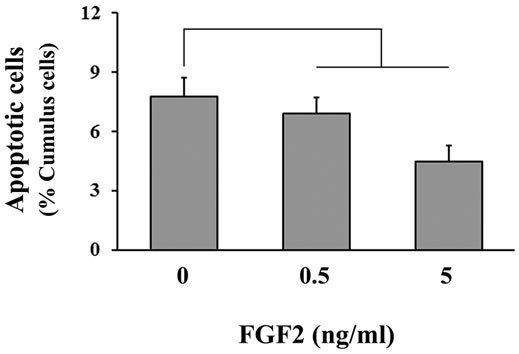
Figure 2. The effect of FGF2 supplementation during IVM on cumulus cell apoptosis. COCs (n = 25 - 35/replicate; 4 replicate studies) were cultured in maturation medium containing FSH and 0, 0.5, 5 or 50 ng/ml FGF2. After 21 h, COCs were fixed and processed to determine the percentage of TUNEL-positive cumulus cells in each COC. A significant difference between controls and both FGF2 treatment groups was detected by using orthogonal contrasts (P = 0.03).
apoptosis-related genes was examined in cumulus cells harvested after 6 and 21 h of maturation. Supplementation with 5 ng/ml FGF2 did not affect the relative abundance of any apoptosis-related transcripts that were examined at each time-point, which included BCL2, XIAP, BAX, BAD and FAS (data not shown).
A final study was completed to determine if FGF2 supplementation altered the expression of selective cumulus-expressed transcripts associated with oocyte competency [30,32,34-36] . Cumulus cells were isolated from COCs cultured in medium containing 0 or 5 ng/ml FGF2 for 6 h or at the completion of IVM. No FGF2-dependent differences in any of the cumulus markers of oocyte competency were detected. Transcripts examined included CTSB, SPRY2, EGFR, FSHR and HAS2 (data not shown).
4. DISCUSSION
This work described whether addition of FGF2 during IVM improves subsequent embryogenesis. Bovine IVM/ IVF/IVC procedures produce embryos that usually are inferior at generating viable offspring than in vivo-derived embryos. Pregnancy rates at day 28 - 32 usually are reduced and subsequent pregnancy losses are increased following transfer of IVP-embryos to recipients at day 7 post-estrus [25,37] . The interest in examining FGF2 as an oocyte competency factor was proposed because it is produced by theca, granulosa and cumulus cells throughout follicuologenesis [21,22] , transcripts for several FGFR isotypes that bind FGF2 preside in cumulus cells and oocytes [6] , and it serves important roles during folliculogenesis [19].
Supplementation with FGF2 during IVM promoted blastocyst formation at day 7 post-IVF. This finding is consistent with recent work examining the role of FGF10 as an oocyte competency factor [6] . However, that study also detected beneficial effects for FGF10 on embryo development at day 3 (% ≥ 8-cell embryos) and day 8 (% advanced blastocysts). These effects were not evident following FGF2 supplementation. Perhaps the less pronounced effects of supplemental FGF2 in this work occurred because cumulus cells produce FGF2 but not FGF10 [20-22] . FGF10 is produced primarily by theca cells, which are absent during IVM [7] . Therefore, it seems reasonable to propose that the impact of supplemental FGF2 could have been masked by endogenous sources of FGF2. Alternatively, FGF2 and 10 act through distinct receptor subtypes [38], and perhaps these differences in biological responses are reflective of this differential receptor usage. Regardless of the reason, observing that both FGF2 and 10 increase blastocyst formation at day 7 is interesting since early blastocyst formation in vitro is associated with increased embryo competency after embryo transfer [39,40] . This implicates both factors as oocyte competency factors.
It remains unclear how FGF2 supplementation impacts oocyte maturation. Increases in polar body extrusion rates were observed when intact COCs were exposed to FGF2 but subsequent fertilization rates were unaffected by FGF2 treatment. Further investigations are needed to describe how FGF2 mediates the timing of meiosis and how this may impact fertilization success.
The exact mechanism(s) used by FGF2 during IVM to impact subsequent embryo development has not been delineated but cumulus cells likely are mediating at least some of the FGF2 effects. Cumulus cells certainly are needed for FGF2-mediated effects on meiosis. Unfortunately the denuded oocyte maturation model could not be used to examine if cumulus cells mediate FGF2 effects on subsequent fertilization and embryo development. Fertilization and development rates were severely compromised in oocytes denuded prior to IVM (data not shown).Cumulus expansion is a good predictor of oocyte competency for fertilization and subsequent embryo development [30,41,42] . However, FGF2 did not affect cumulus expansion and did not affect the relative abundance of transcripts encoding factors that regulate cumulus expansion (EGFR, FSHR, HAS) [32,34,35] . FGF2 also did not affect the relative abundance of other cumulus competency markers (CTSB, SPRY2) [30,36] but improved survival rates of cumulus cells. Cumulus cells undergo spontaneous apoptosis during IVM [43] and FGF2 supplementation limited the percentage of TUNELpositive cumulus cells. The mechanism used by FGF2 to elicit this response remains unknown.
In summary, this work supports the concept that selective factors found within the follicular niche can be used to improve bovine IVP success. Supplementing FGF2 during IVM improves oocyte maturation and blastocyst formation at day 7 post-IVF. The specific mechanisms utilized by FGF2 to improve oocyte competency to form blastocysts was not uncovered but probably is mediated by modifications in cumulus cell activity and/or viability during IVM.
5. ACKNOWLEDGEMENTS
Authors thank William Rembert for his assistance with collecting ovaries and personnel at Central Beef Packing Co. (CenterHill, FL) for their generosity in supplying ovaries for research. Authors also thank Dr. Peter J. Hansen and members of his laboratory for sharing laboratory equipment, space and expertise. Thanks also go to Southeastern Semen Services (Wellborn, FL) for donating bull semen. This project was supported by National Research Initiative Competitive Grant number 2008-35203-19106 from the USDA National Institute of Food and Agriculture.