A Novel Biodegradable Poly (Hydroxybutanedioic Acid-co-2-hydroxypropane-1,2,3-tricarboxylic Acid) Copolymer for Water Treatment Applications ()
1. Introduction
Mineral scale formation in water is a global problem which persists to this day. Mineral scale deposit occurs primarily because of the presence of mineral and salts in the water. Wastewater is continuously discharged from industries such as mining, water treatment sectors, oil refineries, pulp and paper industries, glass and ceramic production industries, nuclear plants, and uranium refineries. This wastewater contains minerals and salts which may contaminate the groundwater. It is well documented that impurities present in feed water and re-circulating water markedly influence the rate of scale deposit [1-2].
Scale formation in cooling water systems shortens the life of equipment. The major causes of industrial water system failures is the deposition of scale on equipment such as heat exchangers and reverse osmosis (RO) on membrane surfaces and this results in increased operational costs [3]. The mineral scale deposits commonly encountered include carbonates, sulfates and phosphates salts of alkaline earth metals.
During the last few decades control of mineral scales formation in industrial waters have been extensively researched. Several reviews that compare the performance of various chemicals and polymers commonly used to control the scale formation are available [4-6]. Various anti-scaling additives have been proposed. Such additives include certain polyphosphates, polyacrylic acids, polymethacrylic acids, polyacrylamides, lignin sulphonic acid, hydrolysed polymaleic anhydride, hydrolysed copolymers as well as their salts. These polymers satisfactorily inhibit scale formation, however, other problems are encountered due to the continued presence of the above mentioned scale inhibitors once their primary function has been achieved. Generally they are difficult to degrade by the action of microorganisms. Toxicity and non-degradation cause major harm to the water systems and the environment if the above mentioned polymers are discharged into the environment. Hence, there is a need to develop a polymer that has both the good scale inhibition and biodegradation properties. Eco-friendly non-toxic biodegradable polymers are excellent candidates for water treatment applications and have been given a lot more attention since the 1970s [7].
Quality and purity are major concerns in water treatment industries. The green polymers are good descaling inhibitors without affecting taste, quality, and purity of water [8,9]. Increasingly stringent environmental restricttions and the need for water conservation, has led to the development of modern, all-organic, non-phosphorus biodegradable polymers for cooling water treatment programs [10]. Over the years a number of investigations were conducted to study the influence of biodegradable polymer on cooling water treatment system [11,12] to minimize the scale deposition [13,14].
In this regard DL-malic acid (DMA) was chosen as a descaling agent because it can be handled safely during the synthesis [15,16]. To further improve their efficacy of scale inhibition citric acid is used as a comonomer. This combinational approach enhances their scale inhibition efficacy.
2. Experimental
2.1. Materials
DL-malic acid (DMA) (Product No. 38798, Minimum assay 99%), citric acid anhydrous (CA) (Product No. 87984, Minimum assay 99%), Calcium chloride (Product No. 20070), Ethylenediaminetetraacetic acid (EDTA), Magnesium chloride (MgCl2) and Sodium bicarbonate (NaHCO3) were purchased from s.d. Fine chemicals, Mumbai, India.
2.2. Synthesis of Poly (DMA-CA) Copolymer
Copolymers were synthesized using the following procedure. Two different weight ratios of both DMA and CA were mixed together to form the copolymer as shown in Table 1. The reactants (DMA and CA) were placed in a ceramic bowl and the monomer mixture was first heated up to 100˚C for 2 h in an oven, then heated to 120˚C for about 10 minutes until the reaction mass partially melted. The temperature was thereafter increased to 130˚C and maintained for 9 h until the mass attained a yellowish colour. Finally, the temperature was increased to 200˚C for 7 h to get a complete conversion to a polymer. The synthesized copolymer was washed with distilled water several times (20 times) at ambient temperature until polymer was free of impurities. The yield of the product was 90% - 95%. The same procedure was followed for the synthesis of poly (DMA) homopolymer. Photographic images of prepared homopolymer and copolymer are shown in Figure 1.
2.3. Experimental Test Method for Calcium Carbonate Scale Inhibition
Scale Test
The polymer (10 ppm) was added to a flask in which calcium chloride, sodium bicarbonate stock solution and 25 ml water was present. Solutions of synthetic cooling wa
Table 1. Feed composition of biodegradable water soluble polymers and their % of CaCO3 inhibition.
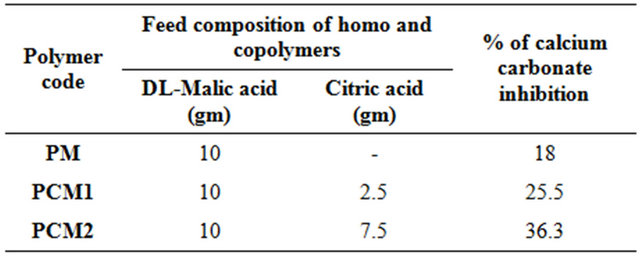
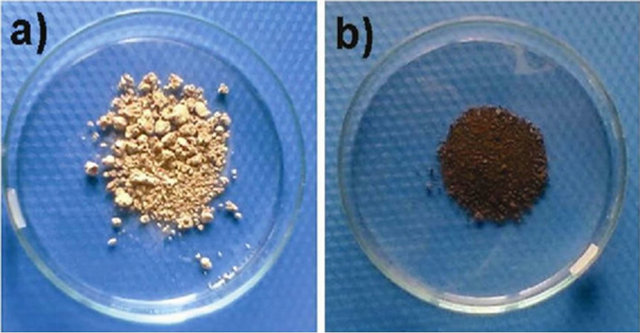
Figure 1. Photograph images of (a) homopolymer (PM) and (b) copolymer (PMC2).
Table 2. Composition of anion and cation brine.
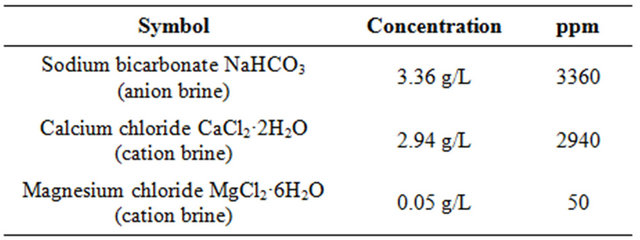
All stock solutions were prepared from analytical reagents and demineralised water (DM) without further purification.
ter (CaCO3 scale) were made by mixing 50 ml of anionbrine to 25 ml of cation brine solution and 25 ml of DM (demineralized) water. Thereafter 25 ml of demineralized water was added to 25 ml of CaCl2 stock solution along with 10 ppm of the polymer solutions (scale inhibitor) in a 250 ml reagent bottle. Thereafter 50 ml of NaHCO3 of stock solution was added to the solution. Another 250 ml reagent bottle was prepared with the same contents expect the polymer solution (scale inhibitor) and was used as the control solution. The pH of both solutions was adjusted to 8.0 by the addition of standard potassium hydroxide solution. Test conditions were set as the follows: without polymer (Blank, hot), with polymer (homopolymer (PM), copolymer (PMC1) and copolymer (PMC2) dose (10 ppm), respectively. The reagent bottles were incubated for 16 hours at 70˚C. After the incubation period, the reagent bottles were removed from the heat source and filtered through a 0.42 micro meter (μm) membrane filters. The filtrate was titrated for calcium using 0.01 molarity (M) EDTA and Eriochrome Black T (EBT) indicator, the percent calcium carbonate inhibition was calculated using the following equation (1) [17]:
(1)
Cai = Calcium ion concentration with the inhibitor.
Cab = Calcium ion concentration in the blank.
Cac = Calcium ion concentration before the test.
3. Characterization
FTIR plots in the range of 500 to 4000 cm−1 was done on a FTIR spectrometer MB3000 Model (Quebec, Canada) using the KBr disk method. The software used was Horizon software from ABB Company. Differential scanning calorimetry (DSC) of poly (DMA) and poly (DMACA) were studied by using a SDT Q 600 DSC instrument (T.A. Instruments-Water LLC, Newcastle, DE 19720, USA) at a heating ramp of 20˚C/min under a constant nitrogen flow (100 ml/min). The samples were heated from 30˚C to 600˚C. The thermogravimetric (TGA) analysis of poly (DMA) and poly (DMA-CA) was evaluated on a SDT Q 600 TGA instrument (T.A. Instruments-Water LLC, Newcastle, DE 19720, USA) at a heating rate of 10˚C/min under a constant nitrogen flow (100 mL/min). The samples were run from 30˚C to 600˚C.
4. Results and Discussion
It was found that the addition of copolymer with higher monomer ratio of citric acid has an effect on the scale inhibition performance. The study demonstrated that the addition of small amounts of aliphatic tricarboxylic acid monomer to malic acid, transforms the polymerization from a solid state to a melt state reaction between 130˚C and 200˚C. In order to understand these copolymers were characterized by the following analysis.
4.1. FTIR Analysis
The FTIR spectra of DMA-CA monomers and poly (malic acid-citric acid) copolymer are shown in Figure 2. The peaks in the range from 3100 cm−1 to 3400 cm−1 are assigned to the fundamental stretching vibration of different -OH groups. The double and single -CO stretch peaks of DMA are located at 1719 and 1272 cm−1, respectively [18,19]. The asymmetric and symmetric -CO stretch bands are situated at 1453 cm−1 and 1376 cm−1 [20]. The spectra of CA shows a broad peak around 3505 cm−1 which indicates -OH stretching. The very distinct peaks at 2773 cm−1 and 2656 cm−1 indicate hydrogen bond formation between -OH groups and carboxylic acid groups. In the spectra two prominent peaks at 1714 cm−1 and 1428 cm−1 is observed from the asymmetrical and
(a)
(b)
Figure 2. FTIR spectra of (a) pure DMA and CA; (b) copolymer (PMC2).
symmetrical stretching of -COO groups respectively. Poly (DMA-CA) spectra shows hydroxyl absorption bands at 3101 cm−1, which correspond to stretching frequency of the OH groups present in poly (malic acidcitric acid). The FTIR spectrum of this high amount of citric acid sample (Figure 2) (data not shown) shows large absorbance at 1618 cm−1 corresponding to carbonyl stretching in the copolymer. Additional peaks were observed at 2681 cm−1, 2530 cm−1 and 1189 cm−1 due to incorporation of CA and DMA (respectively) in copolymer structure. All the above peaks confirm the incorporation of DMA and CA units in the copolymer networks.
4.2. Thermal Analysis
4.2.1. Differential Scanning Calorimetry (DSC)
Figure 3(a) shows the DSC thermograms of the homopolymer (PM) and copolymer (PMC1 and PMC2). DSC of homopolymer and copolymers gives Tg as 300˚C, 288˚C and 280˚C respectively. The glass transition temperature decreases with the increase of citric acid content in copolymer. This is due to the incorporation of citric acid units (aliphatic) which provide more flexibility and chain length to the biodegradable copolymers. Similar observation was reported by Singh et al. [21]. In case of the physical mixture of DMA and CA [PMC2 (Figure 3(c))] shows separate endotherms corresponding to malic acid (137˚C) and citric acid (244˚C) peaks, indicating no