From the Dark Neutron to the Neutron Decay Anomaly and Lithium Cosmologic Problem ()
1. Introduction
In a series of studies [1] [2] [3] on the phenomenology of particles the possibility of representing massive particles as geometric structures of couplings between quantum oscillators of field emerges. The particle thus presents itself with a double aspect: a field of oscillations expressed by a wave equation and a structure at geometric shape of a set of the field oscillators of a field [4]. This obviously extends the descriptive horizon of particles and their interactions, deepening the understanding [5] [6] [7] of the Standard Model (SM). These new aspects suggest that we are facing a new descriptive paradigm of physics: the geometrization of the matter-form. Thanks to this new descriptive paradigm it is possible to formulate the hypothesis that matter occurs in two different forms: an ordinary form, which is easy to detect, and a “dark” form that has difficulty interacting and being detected [8]. Not only that, but the hypothesis of the “dark” form of matter implies a radical and innovative revision of some concepts related to decays or, in general, to interactions, and why not, to the particles themselves. From previous articles [1] [2] [3] [4], we recall the revisitation of the concept of mass, Section 2.1. We show that the mass property derives from the presence of an (additional) transverse coupling between the oscillator lines of a non-massive basic scalar field X. This justifies the hypothesis of structure and defines the new descriptive paradigm of interactions given by the geometric model [8] [9] of particles (PGM). In Section 2.2, it is then highlighted that the additional coupling expresses a generalized “Higgs” field that would give mass to the leptons in any type of interaction: H in the electromagnetic interactions (Hem) and H in the weak ones (Hweak) [10] [11]. This is how the geometric shapes of the H and W bosons are expressed. In Section 2.3 we consider the hypothesis of structure and define the new descriptive paradigm of interactions given by the geometric model of particles (PGM). Always in Section 2.3, we have remarked that the PGM is connected to a revision of fundamental concepts of mass, electric charge and color charge, which find groundwork in the idea of quantum oscillator composed of sub-oscillators. In Section 3.1 we express the geometric shapes also of quarks. Quarks do not need an additional base field, such as H; instead, the quarks (u, d) already have mass because they are defined by the additional coupling between quantum oscillators arranged in “aurea” geometric form or in golden triangles [4] [5] [7] [9]. In this case, the PGM is defined as AGM or the “aureum” geometric model of quarks. Then, in Section 3.2, we can define the geometric structures of nucleons, and, in Section 3.3 also, those of the Deuteron (D) and Tritium (T), seeing these as assemblies of “golden” nucleons. From the geometric description of the fusion reaction [12] of Tritium (T) with Deuteron (D), we can derive the geometric structure of the dark neutron, in Section 4.1. By means of the geometric shape of the dark neutron, its probability P(nd) of emerging in the system (D + T) is calculated and this value is compared (sec. 4.2) with the index of the relative discrepancy (dr)sp between the lifetimes of neutron measured in two different types of experiments: the one in “bottle” [13] [14] [15] and the one in “beam” [16] [17]. Considering the works of Fornal and Grinstein [18] and Gonzalez [19], we find that (dr)sp » P(nd); in this way the hypothesis of structure is strengthened [20]. In Section 4.3 we suggest possible experiments that can reveal the dark neutron. Thanks to the structure equation [9] in Section 4.4, we calculate the mass of the dark neutron, finding m(nd) < m(no), an aspect that prevents it from decaying into a proton. In Section 5.1, the possibility of explaining the asymmetry between matter and antimatter present in our universe [21] is highlighted, originally correlating it with the Asymmetry of Ordinary Matter and Dark (Section 5.2) and the production of dark matter in primordial proto-galaxies [22]. In the final, Section 5.3 the problem of lithium is faced in the primordial phase of the evolution of the universe, that of nucleosynthesis, in the light of the dark neutron hypothesis. It is highlighted that the presence of dark neutrons alters the fusion process that leads to the formation of lithium, unbalancing the probability of lithium 6Li originating to favour of 7Li [23]. We will show that the probability P(nd) of the formation of a dark neutron in fusion reactions is very close to the ratio of the two lithium densities, see the relation: P(nd) » (6Li/7Li).
2. The Particles as Geometric Structures of Coupled Quantum Oscillators
2.1. The Massive Coupling
In previous studies [1] [2] [3] it was noted that the K-G equation describes a massive particle but also a system of pendulums connected by springs. If we put [m = (hω0/c2)], then it is:
(1)
then the “proper” frequency (ω0) is called as massive frequency. The dispersion relationship of waves propagating in (Slab), as described by the Klein-Gordon equation, is:
(2)
We translate this system into a field seen in its representation at coupled oscillators, see Figure 1.
We conjectured that the massive particle-field (YKG) is originated by a “transversal coupling” (T0) between the chains of oscillators of a massless scalar field (X) of base. In this way, the mass is a physical expression of the proper frequency (ω0) related to a particular elastic coupling, which is in addition to the one already existing between the oscillators of (X). This “additional coupling” which
![]()
Figure 1. Massive field as lattice of “pendulums” with springs.
produces the mass in (X), has been referred to as a “massive coupling”. All this can be depicted figuratively as shown in Figure 1. When we observe only the oscillation with frequency (ω0) in all points (x) then we are at rest with the massive particle (
) and X-field do not is involved. Instead when the X-field is involved the wave becomes progressive with frequency (ω) wave length (λ) and represent a massive particle with velocity (v), (see Equation (2)). In Figure 1 the λºλc is the Compton wavelength and represents the space step of massive lattice. By Equation (2) and De Broglie relation one finds the Compton relation: [λc = (h/mc)]. Now we know the mass meaning of a particle: the mass is an additional transversal coupling in a massless scalar field of base. As well it is known, the Equation (1) is derived, by the Lagrange equation, from Lagrange’s function (L) which describes the physics system of Field-Particle, where the Y-Field is the system of quantum oscillators in which the wave-particle propagates. The (£) Lagrange density for Klein-Gordon (Equation (1)), is:
(3)
In the literature the Lagrange function, see (Equation (3)), has an additional term of mass (mY2), but one didn’t say anything about the origin of the mass idea. Now, instead, the additional term of mass (mY2), points out an additional coupling (T0) to field (X) which makes pass from (X) to Y, see Figure 1. Note that the X-field is intrinsic to Y-field. This additional coupling determines into quantum field a “structure” coupled with quantum oscillators: this represents a massive particle. Some clarification is needed. If in field theory we consider a scalar field expressed by a wave function Y(x, y, z, t), we mean that in a point of the space of the laboratory SR it is observed that “something oscillates” and it propagates. The oscillation can be longitudinal or transverse: nobody knows what oscillates. The positives (fathers of QM, early 1900) have denied further investigations that go beyond the wave and corpuscular phenomena (energy quanta of the field). Thus, the whole theory of (quantum) fields can only be expressed in “phenomenological” terms. The Lagrangian function together with its Lagrange equation can only describe phenomena and their correlations (physical quantities). We know, in fact, what happens to particles with a mass, electric charge, color charge, spin, etc. when they interact but we do not know what oscillates in them and what intrinsically are their mass, electric charge, color charge, spin etc.. Now something is being added or rather it is deepening in the theory of quantum fields, see the SM. In the Lagrangian, the mass that appears as an additional term (mY2) to the one that describes the variations of the wave function (¶mY), is no longer a single parameter. Well, this additional coupling determines a coupling structure of the representative oscillators of the field: a particle thus becomes a structure that propagates together with its representative field. Even if we cannot investigate the additional elastic tension T0 which determines the mass, we could instead investigate the coupling structure that the tension T0 realizes. Even if for the moment the structure hypothesis has only a formal expression and lacking an adequate mathematical representation, nevertheless its formulation adds more information in physics that deepens the fundamental concepts of particles and extends knowledge about their behaviours and interactions.
2.2. The Fundamental Massive Bosons
Understanding the mass of a particle as the proper (ω0) frequency of oscillation resulting from the additional transverse coupling in a basic scalar field, may seem to disagree with the idea of mass in the SM, where the Higgs field is the boson that gives the mass to the particles (exactly leptons, weak vector bosons and quarks). However, the same Higgs field has mass and, therefore, we ask us “what other field would give the mass to the Higgs field”. We, instead, assert that if the Higgs field has mass, then it is a lattice-field at additional coupling between the scalar field oscillators not massive (XH) which is more fundamental of the same Higgs field. Thus, thanks to the Higgs field and its additional coupling, the particles that participate in the weak interaction acquire mass, see the Weinberg-Higgs mechanism [11]. This could also happen in the electromagnetic reactions of creating pairs
. In the coupling between two photons, the massive lattice (HemºHg) provides an additional coupling between quantum oscillators of the two photon fields. We may write then:
.
Due to its mass, see Figure 1, the “electromagnetic” Higgs field (Hg) becomes an additional coupling “lattice” that allows electromagnetic energy (photons) to “transform” itself into massive quanta (lepton pair). It is immediately evident that with this new approach to the process of pair creating, a new descriptive paradigm is introduced into the physics of interactions: the interaction agent becomes a lattice-field capable of transforming the particles, from massless to massive or from massive to massive. We specify once again that this argumentation is purely formal and can be useful for didactic purposes. If we look at Figure 1, we can imagine for the Hg boson that it may be represented by Figure 2.
Here, an additional coupling of field oscillators with the spatial dimension of the wavelength λHand submultiples take origin in a massless scalar field X. If we
![]()
Figure 2. The H-lattice at Compton different wavelengths λH.
look at Figure 1 and Figure 2, we can imagine for the W±boson that it may be represented by Figure 3.
2.3. The PGM Model
We note that the (YH, YW) are wave function (massive boson) expressed by the operators (a, a+) with commutator [a, a+](-). The operators (a) represent elementary quantum oscillations. In a theory [10] [11] that sees a particle as a field of coupled oscillators, speaking of structure means considering it as the propagation of an “assembly” of couplings involving several lines of field oscillators [3] [4]. This is possible only if the particle-structure implies a “geometric” form of the couplings that build it. Note that speaking of structure does not mean that the particle is composed: a coupling between field oscillators, even if geometrically structured, makes the particle an elementary unit, that is, it cannot be decomposed into other elementary units. Thus, the (H, W±) boson are elementary units of oscillation associated to a group of coupled field oscillators in geometric form: the structure-particle propagates along two or more field lines, obviously also these coupled, see later in this article. Obviously, to the time independent operators (a0,
), of the wave function we will associate a matrix of operators [((a0)), ((
))] representing the particular coupling that identifies the geometric structure associated to the particle, e.g.
. The atemporal operators (a0,
) carry the information of the type of wave, whether scalar, vector or spinor: they represent annihilation operators and the creation of field quanta that participate in the interactions. Instead, they do not participate in wave phenomena such as interference, diffraction and polarization processes, see operators which dependent of time (a(t), a(t)+). The information of a possible structure of couplings between field oscillators does not contrast with the above because expressed by matrices [((a0)), ((
))]. The particle will thus be described by the Lagrangian formalism as regards the phenomenology of interactions [10] [11]. The structure, on the other hand, is important in the processes of combining the particle's “oscillators” with the interacting particles, the intermediary agents, and in the decay processes because it can give us information on the intrinsic characteristics of the particle and any particles emerging
![]()
Figure 3. The W±-lattice at Compton different wavelengths λw.
in the reactions. Therefore, for the moment we can treat the structures in formal terms without further specifying the mathematical form of the matrix operators [((a0)), ((
))]. We indicate by the acronym (PGM) the Geometric Model of Particles: for the moment this model will be developed by a formal and representative approach didactically useful, but it is intuitive to understand that it can be developed and represented through an adequate mathematical language. The validity of this representative model of particles is based on the possibility of explaining the origin of the fundamental concepts, see mass [4] [7], electric charge [24] [25], color charge [26] and spin, and to deepen the experimental and theoretical aspects of the interaction processes and of particle generation.
3. The “Aureum” Geometric Model (AGM) of Quarks
3.1. Description of the Model
Since quarks also have mass then we need to assign structures of additional couplings of oscillators to them. There is a set of studies on quarks, see Guido [2] [4] [5] [7] and Guido-Bianchi [8], where we have mathematically demonstrated that quarks are triangular “golden” structures of coupled quantum oscillators. It is shown, in fact, that the d-quark will be associated with the basic golden triangle (d) with angles, base (72˚, 72˚), vertex 36˚), while the triangle also known as golden gnomon is associated to the u-quark with angles [base (36˚, 36˚), vertex 108˚)], see Figure 4.
(A, B, C, D, E, F) are vertex oscillators, while all others are junction oscillators (AB, BC, CD, AD) corresponding to gluons with charge of bi-colour [26]. The vector s represents the spin of the fermion quark. X is the propagation axis. To the translation (movement) along the X axis we could add a rotation (the different possible planes of oscillation) of the triangular structure of couplings around the propagation direction; the s-spin of the boson is of eigenvalues: s = ±1/2. Intuitively, it can be assumed that along the propagation axis X, the additional oscillations Ym of the oscillators (A, B, C) and (D, E, F) with frequency ω0 overlap,
in phase, to combined oscillations of the two scalar fields (Xi Ä Xj) or Xg (gluon field), which propagates along the X axis. Here, the Ä is an operation of coupling of Fields (Xi, Xj). The phase relation will be given by Equation (2). The plane of oscillation of the geometric shape rotates around the propagation axis X. The combination of the two oscillations with the rotation of the structure generates a spinor field that will propagate along the X-axis. In this way the additional coupling Ym propagates along the X-axis. What has been said in this section is in the phase of mathematical elaboration for deriving the mathematical formalism suitable for describing the geometric model of quarks, mentioned here. We have referred to this representation of quarks as the “Aureum” Geometric Model of Quarks (AGM) [3] [9]. If quarks are geometric forms of coupling of quantum oscillators (golden triangles (u, d)), then any light hadron composed of quarks (u, d) can be geometrically constructed as an assembly of golden triangles. Recall a geometric property of the golden triangles: into any golden triangle, one can insert two “sub-triangles” (u', d'). In turn, two sub-triangles (u”, d”) are inserted in both u' and d' and so on. These geometric “possibilities” in Quantum Mechanics become possible states of quarks: there are two consequences:
· the structures of quarks (s, c, b, t) are formed with the sub-triangles [2] [5].
· there exists a background lattice {(d,u)} of quarks and “sub-quarks” [4] [7].
In some publications [2] [4] [5] the geometric forms of “heavy” quarks have also been given, such as the quark (s, c, b, t). If we want to be more faithful to physical reality, we consider a background lattice containing “virtual” pairs of quark-antiquarks with the connected “sub-quarks”: {(d, d)Ä(u, u)}. All hadronic particles will propagate within this lattice and here they will interact with each other through quantum exchange. It is no coincidence that Yucawa found between two nucleons there are the pions as intermediary agents. Or even in recent times one speaks of quark “molecules” as lattice particles. By this new descriptive paradigm of hadronic phenomenology, we can explain the phenomenon of “hadronization” and the confinement of quarks. Therefore, the inseparability of quarks or hadronization so as confinement of quarks, would be a consequence of the geometric aspect of the background quark-lattice. The hadronic geometric field will thus be made up of golden quarks all set as in a puzzle. Any hadronic particle will thus be expressed by real “quanta” circulating within a quark-triangle structure, formed by couplings of quantum field oscillators, which in turn propagates along one or more coupled field lines. The potentials of QCD [27] [28] are none other than the diffusion of the quanta-gluons in the background lattice-field. Therefore, there are no “traditional” bond forces between two quarks that make up e.g. the pion. This is represented by a structure given by a coupling of a d quark with a u-quark, both belonging to the background hadron lattice of quarks, in which real quanta circulate (as if they were trapped in the structure) which identify the pion structure and describe its propagation. This structure then propagates along a propagation axis with or without its own rotations. Thus, we explain why the neutron [7] [9] is “embedded” in the pair (d, d) and the masses of the hadrons can be calculated by considering the pairs of bases (d, d). The same happens for all other quarks (s, c, b, t). The didactic value of AGM would be so remarkable: combining the various triangles-quarks it is possible to obtain all the “geometric” representations of hadrons, and possibly also those of the hypothesized “molecules” of quarks [8] [9] and the interactions.
3.2. The Nucleon Structure
The neutron can be built by composite structure of two golden triangles d and a golden triangle u;all three triangles-quarks have a common side lying on the same axis which coincides with the propagation axis of neutron [7] [9]. It is shown that the configurations of the ordinary nucleon, see Figure 5.
Where (A, B, C, D, E) are vertex quantum oscillators. Pay attention that the vertex E is not fixed in the side BC because u-quark rotates around X-axis. The junction oscillators (the sides of the two figures) are represented here by little circles with two colours. However, it should be noted that the junction oscillators can well represent the gluons that bind the vertex oscillators as well as the gluons binding the sides of different quarks. Again, in formal and “didactic” terms, it can be thought that the triangles-quarks rotate around the propagation X-axis, see the vectors s, determining an “orbital” spin that contributes to the total spin of the neutron, see ref. [7] [9]. We note that the description in terms of elasticity (junction oscillators and vertices) of the couplings between quarks is equivalent to that which QED makes of hadrons and their interactions through the potentials associated with the color action of the gluons. In precedents articles we gave the structural equation and calculated them masses [7] [9].
3.3. The Geometric Structure of the Deuteron and Tritium
The first structures in which the neutron binds to other nucleons are Deuteron and Tritium. We compose the Deuteron D (n, p) and the Tritium (n, n, p). Omitting for the moment to draw the pions, which would bind the nucleons, we obtain the configurations of Deuteron and Tritium, see Figure 6.
We note that the proton (in black) is p (d1, u1, u2), while the neutron (in blue) is n (d2, u3, d3). The vectors (si) are the spins. Deuteron is a boson. In Tritium we
![]()
Figure 5. The geometric forms of two nucleons.
![]()
Figure 6. Deuteron configuration and tritium.
note that p (d4, u4, u5), n (d5, u6, d6), n (d7, u7, d8). Tritium, on the other hand, is a fermion. In Figure 6, note that along the X-axis of the tritium there are three aligned d-quarks in spin: this is possible thanks to the color charge, different in the three d-quarks. This aspect tells us that no more than three quarks of the same type can coexist along the propagation X-axis. Let’s now build the system (D +T), see Figure 7.
In Figure 7, note that along the X-axis of the (D + T) there are five d-quark spin vectors (sd) on one side and four u-quark spin vectors (su) on the other side: there is an excess of quarks (fermions) along the X-axis, then we must remove two d-quarks and one of the u-quarks. We can then compose the following exuberant neutron n (d2, u6, d5) with the relative spins (sd2, su6, sd5). The proton of the Deuteron p (d1, u1, u2) can stay along the X-axis and give rise, with the tritium proton, to the He. The surplus neutron is expelled:
. Where no is the ordinary neutron. Now, by observing the figure, the number of possible neutrons can be obtained.
We point out by the notation [(di, dj)(i¹j) Ä (uk)] all possible “neutral” combinations between the d-quark pair (di, dj)(i¹j) and uk-quark, with (i, j) = (1, …, 8) and k = (1, …, 7). The number Nc(d, u, d) of these combinations is given by the following calculation:
(4)
The numerical value of the summation gives us the number of combinations (uk, di, dj) corresponding to the total number of possible neutrons.
The following array scheme may be useful:
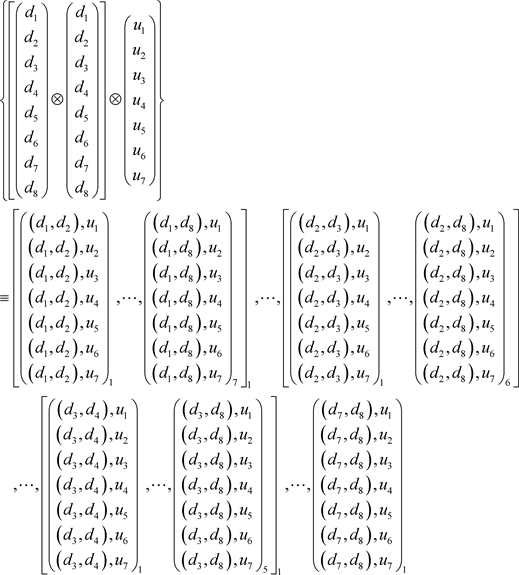
The number of combinations C is
4. The Dark Neutron
4.1. The Structure Anomaly in the of the Neutron
Instead of forming the system (D + T) of Figure 7, it is possible that an anomalous system (D +T)* takes origin, given by the coupling of the Tritium with a Deuteron along the Y axis passing through the AB side of d7 and d5, see Figure 8.
Note that the (X, X', Y) axes are coplanar. There is an interlocking of the quarks d which fixes the pair of quarks (d2, d5). The quark d5 will continue to rotate around the X axis, however dragging with it the d2-quark and together with it the proton (d1, u1, u2). This happens thanks to the present background lattice, {q, q} º {(d, d) Ä (u, u)} coplanar to the axes (XX'): the quanta of the Deuteron can penetrate into the lattice {q, q} and through the gluons of the sides fix the quarks (d2, d5). However, it could happen that the quark d3 couples with the quark d7: the two quarks will detach from their rotation axes and begin to rotate around the Y axis. It could also happen that the d3 drags the quark u3, with which it was bound in the Deuteron, along the Y-axis. In this way, a “strange” neutron is formed propagating and rotating around the Y axis: n (u3, d3, d7)*. Unlike the “ordinary” neutron, this neutron, see Figure 8, has only two quarks (d3, d7) along the propagation Y-axis: the other quark u3, rotating around the X'-axis, is dragged by couple (d3, d7)along Y. Note that u3-quark cannot exchange quanta with any external quarks (belonging to other hadrons) which cross the strange neutron along the Y-axis. This makes the “strange” neutron difficult to interact with other hadrons. The vertex D is insufficient for external energy exchanges (quanta) which instead occur along the whole side AB. Furthermore, the strange neutron is neutral and therefore does not interact electromagnetically. If we highlight that all three quarks of a neutron are involved in its weak decay, it is immediately evident that even the weak decay is compromised because u3-quark is not on the propagation axis. Later we will demonstrate that the strange neutron cannot decay like the ordinary neutron because it has a lower mass than the decay proton, see sec. 3.4. A neutron so made and with difficulty to interact can be a good candidate to become dark matter, see the hypothesis of the dark neutral pion in [8], therefore we will call it “dark” neutron (nd). With d5-quark fixed on the X-axis and d2 fixed on both the X-axis and the X-axis, we will have a new helium (He*) which flows on a train track made
![]()
Figure 8. The anomaly reaction of fusion (D + T)* with a dark neutron.
with two parallel axes (XX'). The remaining quarks rotate around the X-axis and others around the X'-axis. The new neutron (u3, d3, d7) will form the dark neutron nd, see Figure 8. The reaction will be:
. Due to its configuration, the He* will have difficulty to interact with other hadrons, see Figure 8. Furthermore, He* is heavier than ordinary helium He, since the dark neutron which comes emitted in the fusion reaction is lighter than an ordinary neutron. Note that the dark neutron nd (u3, d3, d7) as soon as it forms in (D +T)* detaches from the system and will propagate along the Y axis. The u3-quark is attached to the diagonal AD and rotates around the X’’ axis, while the quarks (d3, d7) will rotate around the Y-axis. There remains the proton p (d1, u1, u2) of the Deuteron which rotates around the X-axis, together with the two quarks d2 and d5, bound together. In this way, Helium He* can thus propagate along the parallel axes XX'. We note that the neutrons obtained by combinations (d2, d5, ui) belonging to He* could also be considered “strange”: this is because it would more difficultly to participate in the various nuclear reactions. It is thus possible that along the Y-axis an anomalous form of neutron can be propagated: nd(u3, d3, d7). Note that we could also have had n (d2, d5, u3) as another dark neutron. Thus, there are only two possibilities for a dark neutron to form but only one of the two would be emitted in the reaction
. We have thus shown that in the anomalous reaction there are two possible combinations that give us a dark neutron, see the Figure 8. Also in this case, in the system (D + T)* the possible neutrons that can be formed with quarks are 196. The probability P (nd)of a dark neutron being emitted will then be:
(5)
A few clarifications must be made: the probability P((D +T)*) of an anomalous reaction forming could depend on the temperature of the neutron gas (P* µT), but once the fusion reaction (D +T)* has taken place the probability of having the emission of the dark neutron will be given by the report 2/196. The production of dark neutrons may have been so remarkable in the hot phases of the universe, the nucleosyntesis phase. Therefore, we can assert that in the nucleosyntesis phase most of that dark matter originated which today would be found in the galactic halos.
4.2. The Relative Discrepancy
Researchers try to explain the reason for the discrepancy in the values of the lifetime τ of the neutrons obtained in two different experimental situations: neutrons (Nb) confined in a container called “bottle” [13] [14] [15] and neutrons in a beam (Nf) [16] [17]. In the first method of measure (in bottle) of the neutron lifetime τbottle, suitably cooled neutrons are stored in a container (bottle) for a Δttime comparable to their lifetime. After, the remaining neutrons are counted (nrb) by means of the exponential function exp(−t/τn), during the next their decay [18] [19]. The expectation was to detect in the two experiments the same lifetime (τb = τf); instead, the opposite was experimentally verified (τb < τf). We then ask the reason for the experimental result given by (τb < τf). We consider the difference [Δτn= (<τf> - <τb>)], see the anomaly, and we calculate (Δτ/ < τf>), which represents the value of “relative discrepancy” (dr). We take as reference value of neutron lifetime in the bottle that is obtained by average of values τb (Gonzalez) [18] and τb (Fornal and Grinstein) [19]:
, while for the average life in the beam we consider the average value quoted by F-G but relative to the experiment of Yue [16], [τf = (887.7 ± 1.2[stat])s].
We can find the experimental value of relative discrepancy value:
(6)
The result is that the experimental relative discrepancies (dr)expis coincident, within the error intervals to the probability, see the Equation (5) to have a dark neutron in the fusion reaction (D+T): P(nd)»(dr)exp. Evidently the two numbers are the expression of the same physical quantity, an index of the anomaly of the neutron decay. We can define the probability P(nd), given the context in which it was calculated, as the theoretical relative discrepancy (dr)th, while the one obtained from the measurement data, we define the experimental relative discrepancy (dr)exp. The result is that the two relative discrepancies, the experimental one (dr)expand the theoretical one (dr)thin the dark neutron model, coincide within the error intervals: (dr)th»(dr)exp.
4.3. Dark Neutron Detection Experiment
The first experiment that we could carry out to detect the presence of dark neutrons could be just that of fusion of Deuteron with Tritium, (D +T). If in the fusion process a dark neutron emerges, we should not detect any ordinary neutron:
.
We would have the formation of a He* which in some properties could differ from the “ordinary” He, without emission of a neutron. Therefore, in fusion processes (D + T) could miss some neutrons. The second experiment could be made in bottle. In the bottle, dark neutrons should remain which have not decayed and which are not subsequently counted in nr. We can then think of detecting them in a way: directing a beam of pions on the bottle. Some dark neutrons can interact with a pion and determine a particular reaction that easily identifies the dark neutron (
). This reaction would be distinguishable from the ordinary reaction (
), because the mass of the dark neutron is different from that of the ordinary neutron no: m(nd) < m(no). Then, we direct some pions after counting the remaining neutrons nr; if we detect some gamma ray g production with proton, it means that some pion has encountered a dark neutron and managed to interact with it.
4.4. The Dark Neutron Mass
Using the “Aureum” Geometric Modell (AGM), see Section 2.1, we could take into account the structure equation [8] [9] of dark neutron, which is built on the geometric representation of particle, see Figure 8. We can represent the dark neutron also in the following way, Figure 9.
Note u-quark in rotation around to side BC and the pair (d3, d3). This pair represents the field of virtual quarks that “dresses” the mass of a hadron, as described in strong interactions, see Section 2.1. Note that the pairs (di,di)virtuals can be assembled, fitting together, like pieces of a “puzzle”. The geometric structure of the quarks causes the pairs (di, di)virtuals to fit together and form a background lattice of the universe inside to one given by {(d, d) Ä (u, u)}. Within this lattice the hadronic particle-fields propagate as wave fronts of “field lines”, each with its coupling structure that identifies the particle.
The AGM model is so physically equivalent to the field theory of SM. The structure equation allows you to calculate the mass of the dark neutron, using the same procedure by which we have calculated the mass of light mesons [6] [20] and nucleons [7] [8] [9] for the dark neutron we have:
(7)
Here, the symbol Ä indicates the elastic coupling operation which can admit the interpenetration of quarks during their rotations, in the same way as happens in waves when they cross. The interpenetration is thus a purely quantum “wave” aspect: the quarks overlap as happens in the waves represented in QM by the Y states. The waves cross each other and at the points where they cross then they overlap (Y= Y1+Y2) or the oscillations are composed. The quarks, seen as geometric structures of coupled oscillators, are crossed along the sides (chains of junction gluons) by oscillations: the interpenetration of two quarks (q1,q2) implies that the oscillations (Y1,Y2) in their respective sides when they cross then they overlap like waves and cross each other resuming their “path” along the joining sides. The symbol Ä indicates the combination of two operations: Ä º (Ä, Å), where Å indicates the dynamic coupling operation between quarks (exchange of energy quanta). To the structure equation we associate the spin structure equation (the spin distributions into internal quarks), which in the case of the dark neutron becomes:
![]()
Figure 9. The dark neutron configuration.
(8)
In accordance with the procedure for calculating the mass of an ordinary nucleon in AGM, see ref. [7], we consider the “elastic” vertices of the geometric figure:
.
The number of “elastic” vertices is: N(Vel) º Nel = 7. This number represents an elastic characteristic of the structure of quarks: more vertices there are and more elastic tension (Kel) there is into structure.
The elastic tension does increase the oscillation frequency in an elastic system, see the harmonic oscillator: [ω = (Kel/Min)1/2].
Instead, the inertial characteristic (Min) of the structure is connected to the N' number of couplings between two adjacent quarks and referred to the sides of the structure, here are the sides (AC, BC):
It follows: N'(Min) º Nin = 8. The coefficient kd is:
(9)
To elaborate the structure equation the following algebraic relationship is used [7]:
We obtain:
(10)
The mass calculation uses Fm-function applied to structure Equation (7), see [7] [9]. Then, it is:
(11)
where
with
(12)
In all two cases, the two quarks (d1, d2) cannot interpenetrate each other, because they have parallel spin, see the Equation (6): then, it is (d1Äd2) = 0 and, thus, [m(d1Äd2) = 0], see Table 2 in ref [9]. It follows, see Equation (12):
(13)
where the relative mass of two interpenetration quarks is given by:
coupling while in dynamic interaction it is instead:
.
Note that the u-quark is not interpenetrating with the d2-quark. However, u-quark with the quark d2 it has a vertex C in common where it can exchange some bond “gluons”, so we can still associate a mass value to system (uÄd2) as if they are interpenetrating. Therefore, we have
.
Thus, it is:
(14)
Then:
(15)
Having [mu = (53.31) MeV, md = (86.26) MeV] it follows m (1, u>)» (69.79) MeV and thus:
It follows
(16)
The matrix of mass defect also admits the elements Aii ¹ 0. Then, see Table 1.
We calculate the value of table:
(17)
Recall that:
,
,
For the spin (s = 1/2), see the table of the neutron spin, we can have the term R':
(18)
Then, it is:
![]()
Table 1. The couplings with the interaction of quarks in a neutron.
(19)
The pair (d3, d3) in the structure of neutron shields the electromagnetic interactions between quarks, therefore it weakens the mass defect. The presence of the pair d-quark along diagonal, see Figure 9, splits all mass defects, see the ref. [7] [9], then it follows:
(20)
Like the neutron being explicit the elasticity parameter kd, it is, see the Equation (8):
(21)
Thus, it is:
(22)
The difference between m(nd) and m(no) is Δmod = (939.57 - 936.63) MeV= (2.94) MeV. The mass of the dark neutron is even smaller than that of the proton.
5. The Consequences
5.1. The Origins of Matter-Antimatter Asymmetry
The idea of a dark nucleon, although paradoxical, opens the way to a decisive clarification on the nature of dark matter. The geometric structure of the dark neutron nd, see Figure 9, makes it very low probability of interaction with ordinary matter. This is because the u-quark is not attached to the diagonal BD which lies on the propagation axis X', where the interacting particles exchange energy quanta. Therefore u-quark does not couple with any other quarks of other particles, except with quarks (d3, d7) which belong to the same dark neutron. This aspect will not allow the dark neutron to annihilate itself with ordinary and dark antineutrons. The dark neutron does not participate in electromagnetic interactions because it is electrically neutral. Finally, the mass value of the dark neutron (m(nd)< m(no)) and the mass difference with the ordinary one (Δmod = (2, 94) MeV) prevents it from decaying into a proton. The dark neutron is thus stable. The interaction difficulty and of annihilation could explain the asymmetry between matter and antimatter in the universe [21]. The first dark particle that originated (for example from a fusion reaction (D +T)) determined the matter-antimatter asymmetry because it has produced an excess of the antagonist ordinary particles: if instead of an ordinary antineutron there was a first dark antineutron (for example from a fusion reaction
, which could not then annihilate a dark neutron or an ordinary neutron, then over time an excess of matter at the expense of antimatter it was formed:[(N)n, (N − 1)n + nd].
5.2. The Asymmetry of Ordinary Matter and Dark
There may be a connection between matter-antimatter asymmetry and the production of excess dark hadronic matter. We have seen in the previous sec. that the dark neutrons formed can couple without annihilating themselves with the dark and ordinary antineutrons (nd, nd), (nd, no). On the other hand, the same geometric structure allows the aggregation of dark matter into “clusters” of dark neutrons interspersed with dark neutral pions. Thus, clusters of ordinary antimatter aggregated with dark antimatter and dark matter are so formed. These clusters do not annihilate with ordinary matter and do not participate in nuclear interactions; they can only aggregate into super-aggregates of clusters that are dynamically less mobile than neutrons. The ordinary and dark antimatter aggregated with dark matter will thus converge in slow and cold densities, which in the rotational dynamics of the proto-galaxies will be centrifuged outwards [22], determining the halos of dark matter and dark and ordinary antimatter.
5.3. The Lithium Cosmologic Problem
The fusion reaction between Tritium and Helium produces the isotope of Lithium 7Li; we'll have:
(23)
If we did instead
we would get a “regular” lithium 6Li.
Instead, it turns out that 7Li is much more abundant in the universe than 6Li [23]; in fact it is found that 6Li = (1%) 7Li.
We point out that the first to be formed during the nucleosynthesis phase is Deuteron, then Tritium, followed by Helium and finally Lithium.
It is known that is:
In AGM we have demonstrated it “geometrically-mind”, see Section 3.3.
This neutron n can be made available to form another Deuteron D(n,p) that is
as well as other Tritium or He:
,
,
This D can then bind to the regular He and determine the 6Li, see reaction R*:
(24)
We refer to this reaction as R* and He* as Helium with a dark neutron inside it.
The 6Li should be abundant in the nucleosynthesis phase. However, this is not the case: the 7Li would be more abundant. In the innovative hypothesis that the dark neuter exists, we have seen that the following reaction can occur:
In Section 4.1, see Equation (5), we have calculated that the probability of originating a dark neutron in the above fusion reaction is given by
In this case the nd cannot be available to form a D. We will have 1% less Deuterons.
Therefore, the presence of nd makes Deuteron less dense than Tritium T and therefore there would be more presence of Tritium and the most frequent reaction would be the one that gives us 7Li and not 6Li, see Equation (23). In fact, the reaction R*, Equation (24), is not activated because an initial Deuteron is missing.
6. Conclusion
The presence of the anomaly has made it possible to detect that dark matter and ordinary matter are two sides of the same coin: matter. This was possible because we moved from a perspective of point-like particles (quanta) quantum expression of fields to a perspective of particles structures of oscillators coupled with geometric shapes. This shift in perspective opens the way to a new descriptive paradigm in particle physics. We could also speak of a second attempt at the geometrization of physics: if the first concerned the geometrization of space-time, the second is that of the geometrization of particle-fields.
Conflicts of Interest
The authors declare no conflicts of interest regarding the publication of this paper.
References
- 1. Weinberg, S. (1977) The Problem of Mass. Transactions of the New York Academy of Sciences, 38, 185-201. https://doi.org/10.1111/j.2164-0947.1977.tb02958.x
- 2. Guido, G. (2017) About Structure of the Quarks. Hadronic Journal, 40, 221-253. http://dx.doi.org/10.29083/HJ.40.03.2017
- 3. Crawford Jr., F.S. (1965) Waves. McGraw-Hill, New York.
- 4. Guido, G. (2019) The Bare and Dressed Masses of Quarks in Pions via the of Quarks’ Geometric Model. Journal of High Energy Physics, Gravitation and Cosmology, 5, 1123-1149. https://doi.org/10.4236/jhepgc.2019.54065
- 5. Guido, G. (2017) Regarding the Structure of Quarks and Hadrons. Hadronic Journal, 40, 187-219. http://dx.doi.org/10.29083/HJ.40.02.2017
- 6. Guido, G. (2020) The Theoretical Value of Mass of the Light ŋ-Meson via the of Quarks’ Geometric Model. Journal of High Energy Physics, Gravitation and Cosmology, 6, 368-387. https://doi.org/10.4236/jhepgc.2020.63030
- 7. Guido, G. (2021) Theoretical Spectrum of Mass of the Nucleons: New Aspects of the QM. Journal of High Energy Physics, Gravitation and Cosmology, 7, 123-143. https://doi.org/10.4236/jhepgc.2021.71006
- 8. Bianchi, A. and Guido, G. (2021) A New Hypothesis on the Dark Matter. Journal of High Energy Physics, Gravitation and Cosmology, 7, 572-594. https://doi.org/10.4236/jhepgc.2021.72033
- 9. Guido, G. (2020) A New Descriptive Paradigm in the Physics of Hadrons, and Their Interactions. Global Journal of Science Frontier Research (A): Physics and Space Science, 20, 41-50. https://doi.org/10.34257/GJSFRAVOL20IS13PG41
- 10. Morpurgo, G. (1992) Introduzione alla fisica delle particelle. Zanichelli, Milan.
- 11. Quigg, C. (1997) Gauge Theories of the Strong, Weak, and Electromagnetic Interactions. Perseus Westview Press, Boulder.
- 12. Ito, T.M., et al. (2018) Performance of the Upgraded Ultracold Neutron Source at Los Alamos National Laboratory and Its Implication for a Possible Neutron Electric Dipole Moment Experiment. Physical Review C, 97, Article ID: 012501. https://doi.org/10.1103/PhysRevC.97.012501
- 13. Arzumanov, S., Bondarenko, L., Chernyavsky, S., et al. (2015) A Measurement of the Neutron Lifetime Using the Method of Storage of Ultracold Neutrons and Detection of Inelastically Up-Scattered Neutrons. Physics Letters B, 745, 79-89. https://doi.org/10.1016/j.physletb.2015.04.021
- 14. Morris, C.L., Adamek, E.R., Broussard, L.J., et al. (2017) A New Method for Measuring the Neutron Lifetime Using an in Situ Neutron Detector. Review of Scientific Instruments, 88, Article ID: 222501. https://doi.org/10.1063/1.4983578
- 15. Serebrov, A.P., Kolomensky, E.A., Fomin, A.K., et al. (2018) Neutron Lifetime Measurements with the Big Gravitational Trap for Ultracold Neutrons. Physical Review C, 97, Article ID: 055503.
- 16. Yue, A.T., Dewey, M.S., Gilliam, D.M., et al. (2013) Improved Determination of the Neutron Lifetime. Physical Review Letters, 111, Article ID: 222501. https://doi.org/10.1103/PhysRevLett.111.222501
- 17. Hoogerheide, S.F. (2019) Progress on the BL2 Beam Measurement of the Neutron Lifetime. EPJ Web of Conferences, 219, Article ID: 03002.https://doi.org/10.1051/epjconf/201921903002
- 18. Fornal, B. and Grinstein, B. (2018) Dark Matter Interpretation of the Neutron Decay Anomaly. Physical Review Letters, 120, Article ID: 191801. https://doi.org/10.1103/PhysRevLett.120.191801
- 19. Gonzalez, F.M., Fries, E.M., Cude-Woods, C., et al. (2021) Improved Neutron Lifetime Measurement with UCNτ. Physical Review Letters, 127, Article ID: 162501. https://doi.org/10.1103/PhysRevLett.127.162501
- 20. Guido, G. (2020) The Theoretical Value of Mass of the Light ŋ-Meson via the of Quarks’ Geometric Model. Journal of High Energy Physics, Gravitation and Cosmology, 6, 368-387. https://doi.org/10.4236/jhepgc.2020.63030
- 21. Casaus, J. (2009) The AMS-02 Experiment on the ISS. Journal of Physics: Conference Series, 171, Article ID: 012045. https://doi.org/10.1088/1742-6596/171/1/012045
- 22. Trimble, V. (1994) Proceedings of the 1st International Symposium on Sources of Dark Matter in the Universe, Los Angeles, California. World Scientific, Singapore.
- 23. Mathews, G.J., Kedia, A., Sasankan, N., et al. (2020) Cosmological Solutions to the Lithium Problem. JPS Conference Proceedings, 31, Article ID: 011033. https://doi.org/10.7566/JPSCP.31.011033
- 24. Guido, G. (2014) The Substructure of a Quantum Field-Oscillator. Hadronic Journal, 37, 83.
- 25. Guido, G. (2012) The Substructure of a Quantum Oscillator Field. arXiv:1208.0948.
- 26. Guido, G. (2019) The Origin of the Color Charge into Quarks. Journal of High Energy Physics, Gravitation and Cosmology, 5, 1-34. https://doi.org/10.4236/jhepgc.2019.51001
- 27. Bazavov, A., Toussaint, D., Bernard, C., et al. (2010) Nonperturbative QCD Simulations with 2+1 Flavors of Improved Staggered Quarks. Reviews of Modern Physics, 82, 1349-1417. https://doi.org/10.1103/RevModPhys.82.1349
- 28. Ishikawa, T., Aoki, S., Fukugita, M., et al. (2008) Light Quark Masses from Unquenched Lattice QCD CP-PACS and JLQCD Collaborations. Physical Review D, 78, Article ID: 011502. https://doi.org/10.1103/PhysRevD.78.011502