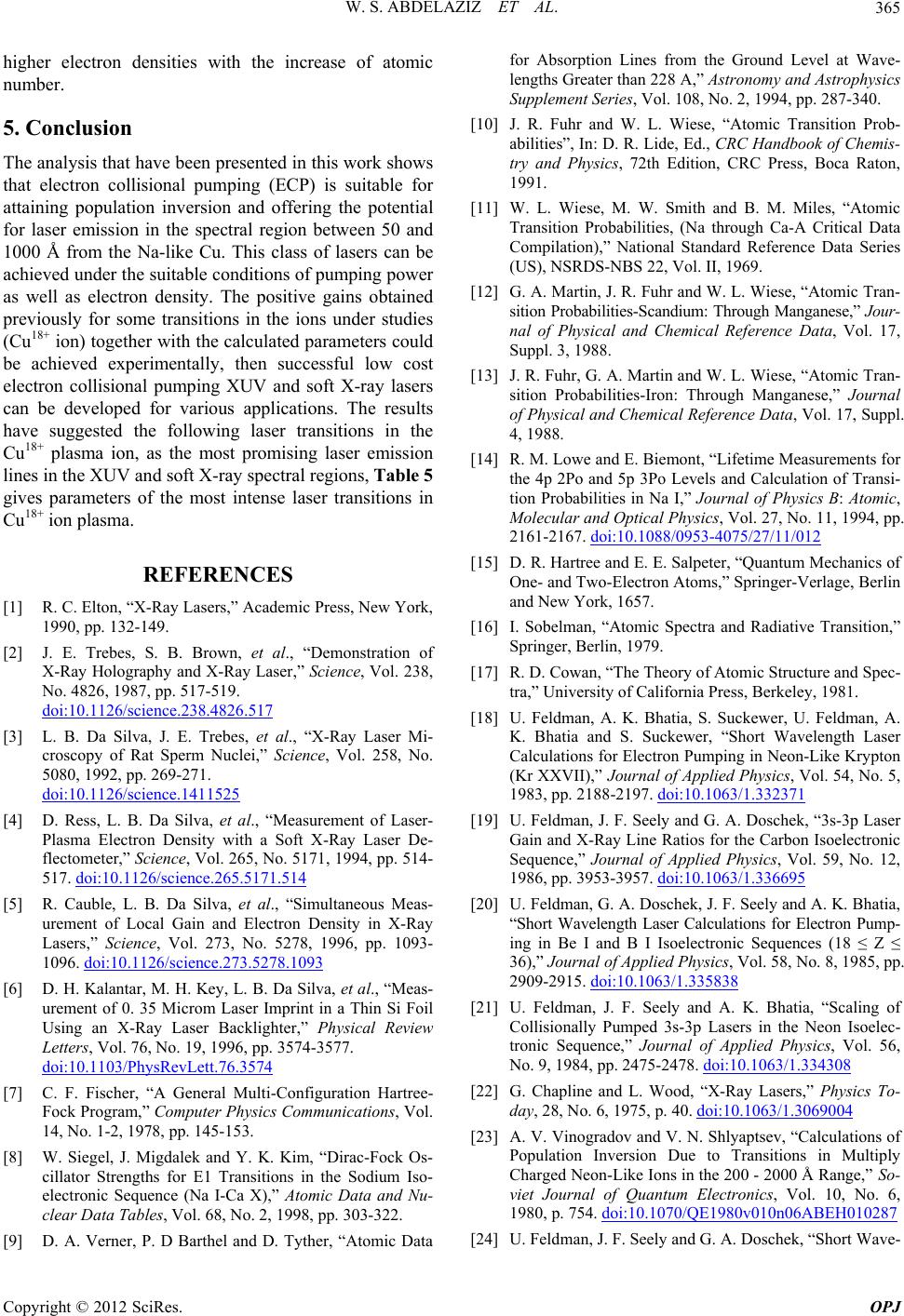
W. S. ABDELAZIZ ET AL. 365
higher electron densities with the increase of atomic
number.
5. Conclusion
The analysis that have been presented in this work shows
that electron collisional pumping (ECP) is suitable for
attaining population inversion and offering the potential
for laser emission in the spectral region between 50 and
1000 Å from the Na-like Cu. This class of lasers can be
achieved under the suitable conditions of pumping power
as well as electron density. The positive gains obtained
previously for some transitions in the ions under studies
(Cu18+ ion) together with the calculated parameters could
be achieved experimentally, then successful low cost
electron collisional pumping XUV and soft X-ray lasers
can be developed for various applications. The results
have suggested the following laser transitions in the
Cu18+ plasma ion, as the most promising laser emission
lines in the XUV and soft X-ray spectral regions, Table 5
gives parameters of the most intense laser transitions in
Cu18+ ion plasma.
REFERENCES
[1] R. C. Elton, “X-Ray Lasers,” Academic Press, New York,
1990, pp. 132-149.
[2] J. E. Trebes, S. B. Brown, et al., “Demonstration of
X-Ray Holography and X-Ray Laser,” Science, Vol. 238,
No. 4826, 1987, pp. 517-519.
doi:10.1126/science.238.4826.517
[3] L. B. Da Silva, J. E. Trebes, et al., “X-Ray Laser Mi-
croscopy of Rat Sperm Nuclei,” Science, Vol. 258, No.
5080, 1992, pp. 269-271.
doi:10.1126/science.1411525
[4] D. Ress, L. B. Da Silva, et al., “Measurement of Laser-
Plasma Electron Density with a Soft X-Ray Laser De-
flectometer,” Science, Vol. 265, No. 5171, 1994, pp. 514-
517. doi:10.1126/science.265.5171.514
[5] R. Cauble, L. B. Da Silva, et al., “Simultaneous Meas-
urement of Local Gain and Electron Density in X-Ray
Lasers,” Science, Vol. 273, No. 5278, 1996, pp. 1093-
1096. doi:10.1126/science.273.5278.1093
[6] D. H. Kalantar, M. H. Key, L. B. Da Silva, et al., “Meas-
urement of 0. 35 Microm Laser Imprint in a Thin Si Foil
Using an X-Ray Laser Backlighter,” Physical Review
Letters, Vol. 76, No. 19, 1996, pp. 3574-3577.
doi:10.1103/PhysRevLett.76.3574
[7] C. F. Fischer, “A General Multi-Configuration Hartree-
Fock Program,” Computer Physics Communications, Vol.
14, No. 1-2, 1978, pp. 145-153.
[8] W. Siegel, J. Migdalek and Y. K. Kim, “Dirac-Fock Os-
cillator Strengths for E1 Transitions in the Sodium Iso-
electronic Sequence (Na I-Ca X),” Atomic Data and Nu-
clear Data Tables, Vol. 68, No. 2, 1998, pp. 303-322.
[9] D. A. Verner, P. D Barthel and D. Tyther, “Atomic Data
for Absorption Lines from the Ground Level at Wave-
lengths Greater than 228 A,” Astronomy and Astrophysics
Supplement Series, Vol. 108, No. 2, 1994, pp. 287-340.
[10] J. R. Fuhr and W. L. Wiese, “Atomic Transition Prob-
abilities”, In: D. R. Lide, Ed., CRC Handbook of Chemis-
try and Physics, 72th Edition, CRC Press, Boca Raton,
1991.
[11] W. L. Wiese, M. W. Smith and B. M. Miles, “Atomic
Transition Probabilities, (Na through Ca-A Critical Data
Compilation),” National Standard Reference Data Series
(US), NSRDS-NBS 22, Vol. II, 1969.
[12] G. A. Martin, J. R. Fuhr and W. L. Wiese, “Atomic Tran-
sition Probabilities-Scandium: Through Manganese,” Jour-
nal of Physical and Chemical Reference Data, Vol. 17,
Suppl. 3, 1988.
[13] J. R. Fuhr, G. A. Martin and W. L. Wiese, “Atomic Tran-
sition Probabilities-Iron: Through Manganese,” Journal
of Physical and Chemical Reference Data, Vol. 17, Suppl.
4, 1988.
[14] R. M. Lowe and E. Biemont, “Lifetime Measurements for
the 4p 2Po and 5p 3Po Levels and Calculation of Transi-
tion Probabilities in Na I,” Journal of Physics B: Atomic,
Molecular and Optical Physics, Vol. 27, No. 11, 1994, pp.
2161-2167. doi:10.1088/0953-4075/27/11/012
[15] D. R. Hartree and E. E. Salpeter, “Quantum Mechanics of
One- and Two-Electron Atoms,” Springer-Verlage, Berlin
and New York, 1657.
[16] I. Sobelman, “Atomic Spectra and Radiative Transition,”
Springer, Berlin, 1979.
[17] R. D. Cowan, “The Theory of Atomic Structure and Spec-
tra,” University of California Press, Berkeley, 1981.
[18] U. Feldman, A. K. Bhatia, S. Suckewer, U. Feldman, A.
K. Bhatia and S. Suckewer, “Short Wavelength Laser
Calculations for Electron Pumping in Neon-Like Krypton
(Kr XXVII),” Journal of Applied Physics, Vol. 54, No. 5,
1983, pp. 2188-2197. doi:10.1063/1.332371
[19] U. Feldman, J. F. Seely and G. A. Doschek, “3s-3p Laser
Gain and X-Ray Line Ratios for the Carbon Isoelectronic
Sequence,” Journal of Applied Physics, Vol. 59, No. 12,
1986, pp. 3953-3957. doi:10.1063/1.336695
[20] U. Feldman, G. A. Doschek, J. F. Seely and A. K. Bhatia,
“Short Wavelength Laser Calculations for Electron Pump-
ing in Be I and B I Isoelectronic Sequences (18 ≤ Z ≤
36),” Journal of Applied Physics, Vol. 58, No. 8, 1985, pp.
2909-2915. doi:10.1063/1.335838
[21] U. Feldman, J. F. Seely and A. K. Bhatia, “Scaling of
Collisionally Pumped 3s-3p Lasers in the Neon Isoelec-
tronic Sequence,” Journal of Applied Physics, Vol. 56,
No. 9, 1984, pp. 2475-2478. doi:10.1063/1.334308
[22] G. Chapline and L. Wood, “X-Ray Lasers,” Physics To-
day, 28, No. 6, 1975, p. 40. doi:10.1063/1.3069004
[23] A. V. Vinogradov and V. N. Shlyaptsev, “Calculations of
Population Inversion Due to Transitions in Multiply
Charged Neon-Like Ions in the 200 - 2000 Å Range,” So-
viet Journal of Quantum Electronics, Vol. 10, No. 6,
1980, p. 754. doi:10.1070/QE1980v010n06ABEH010287
[24] U. Feldman, J. F. Seely and G. A. Doschek, “Short Wave-
Copyright © 2012 SciRes. OPJ